Exploring the fundamental principles that shape our universe.
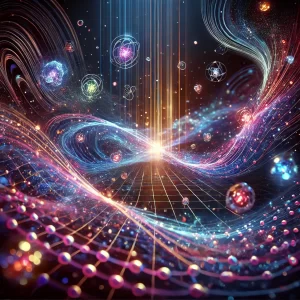
1. Wave-Particle Duality
One of the most surprising discoveries in quantum mechanics is that subatomic particles, such as electrons and photons (particles of light), exhibit a dual nature—they can behave as both particles and waves, depending on how they are observed. This phenomenon is demonstrated by the double-slit experiment, where an electron can interfere with itself like a wave, but when measured, it behaves like a particle. This duality challenges our classical understanding of nature.
2. Quantum Superposition
In classical physics, an object has a defined state. For instance, a ball is in a specific place at a given time. In quantum mechanics, however, particles can exist in a superposition of multiple possible states simultaneously. A well-known example is an electron in an atom: before measuring its position, it can be in several locations at once. This idea defies classical intuition. The famous thought experiment, Schrödinger’s cat, illustrates this paradox where a cat in a box can be both alive and dead until it is observed.
3. Quantum Entanglement
Quantum entanglement is one of the most fascinating and mysterious phenomena in quantum physics. When two particles become entangled, the state of one (in terms of properties like spin or polarization) instantaneously affects the state of the other, regardless of the distance between them. Albert Einstein referred to this as «spooky action at a distance,» as it seems to defy the principle that information cannot travel faster than the speed of light. Despite its paradoxical nature, entanglement has been experimentally verified and is fundamental to technologies like quantum cryptography and quantum computing.
4. Heisenberg’s Uncertainty Principle
Formulated by Werner Heisenberg, the uncertainty principle states that there is a fundamental limit to how precisely we can know certain pairs of properties of a particle simultaneously. For example, the more precisely we know the position of a particle, the less precisely we can know its momentum (or velocity), and vice versa. This uncertainty is not due to a lack of precision in our measuring tools but an inherent property of quantum systems.
5. Wavefunction and Collapse
In quantum mechanics, the state of a quantum system is described by a wavefunction, which encodes all the possible information about the system, but in probabilistic terms. The wavefunction provides the probabilities of a system being in a particular state, but it doesn’t define with certainty what will happen until a measurement is made. Upon measuring a property of the system, such as the position of a particle, the wavefunction «collapses,» and the system reduces to a definite state.
6. Pauli Exclusion Principle
The Pauli exclusion principle, formulated by Wolfgang Pauli, explains why electrons in an atom occupy different energy levels. Two fermions (particles like electrons that obey this principle) cannot occupy the same quantum state simultaneously. This principle is responsible for the structure of atoms and, therefore, the vast diversity in chemical elements and materials.
7. Quantum Computing
Unlike classical computers, which use bits to process information (each bit being either 0 or 1), quantum computers use qubits. Qubits exploit quantum superposition, allowing them to be in multiple states (0 and 1) simultaneously. This gives quantum computers exponentially faster processing power for certain types of problems. Additionally, entanglement between qubits can generate complex correlations that are not possible in classical systems. Quantum computing has the potential to revolutionize fields like material simulation, cryptography, optimization, and artificial intelligence.
8. Interpretations of Quantum Mechanics
One of the deep debates in quantum mechanics revolves around how to interpret its probabilistic nature. Here are a few important interpretations:
- Copenhagen Interpretation: The standard interpretation, promoted by physicists like Niels Bohr, suggests that the quantum wavefunction is purely probabilistic, and only when a measurement is made does the quantum system «collapse» into a defined state.
- Many-Worlds Interpretation: Proposed by Hugh Everett, this interpretation suggests that all possible outcomes of a quantum measurement actually occur, but in parallel universes. In other words, every time a quantum event is measured, the universe «splits» into multiple universes, each containing a different result.
- Hidden Variables Theories: These theories suggest that there is additional information in nature that we have not yet discovered. If we knew this hidden information, we could predict quantum outcomes with certainty, eliminating the need for probabilistic interpretations. This view was supported by Einstein, though experimental evidence has generally favored the randomness inherent in quantum systems.
Philosophical and Technological Implications
Quantum mechanics not only drives technological innovations but also poses deep philosophical questions about the nature of reality, the role of the observer, and the possibility of multiple universes. Technologies like quantum sensors, quantum cryptographic algorithms, and quantum teleportation are still in their early stages but hold the potential to reshape the future of science and technology.
In conclusion, quantum physics describes a world fundamentally different from our everyday experiences. Its principles might seem counterintuitive and strange, but they have been rigorously confirmed through experiments and serve as the foundation for many modern technologies.